Should Molecular Biologists Pay Attention to This Important Neuroscience Data?
An interview with Fredrik Johansson.
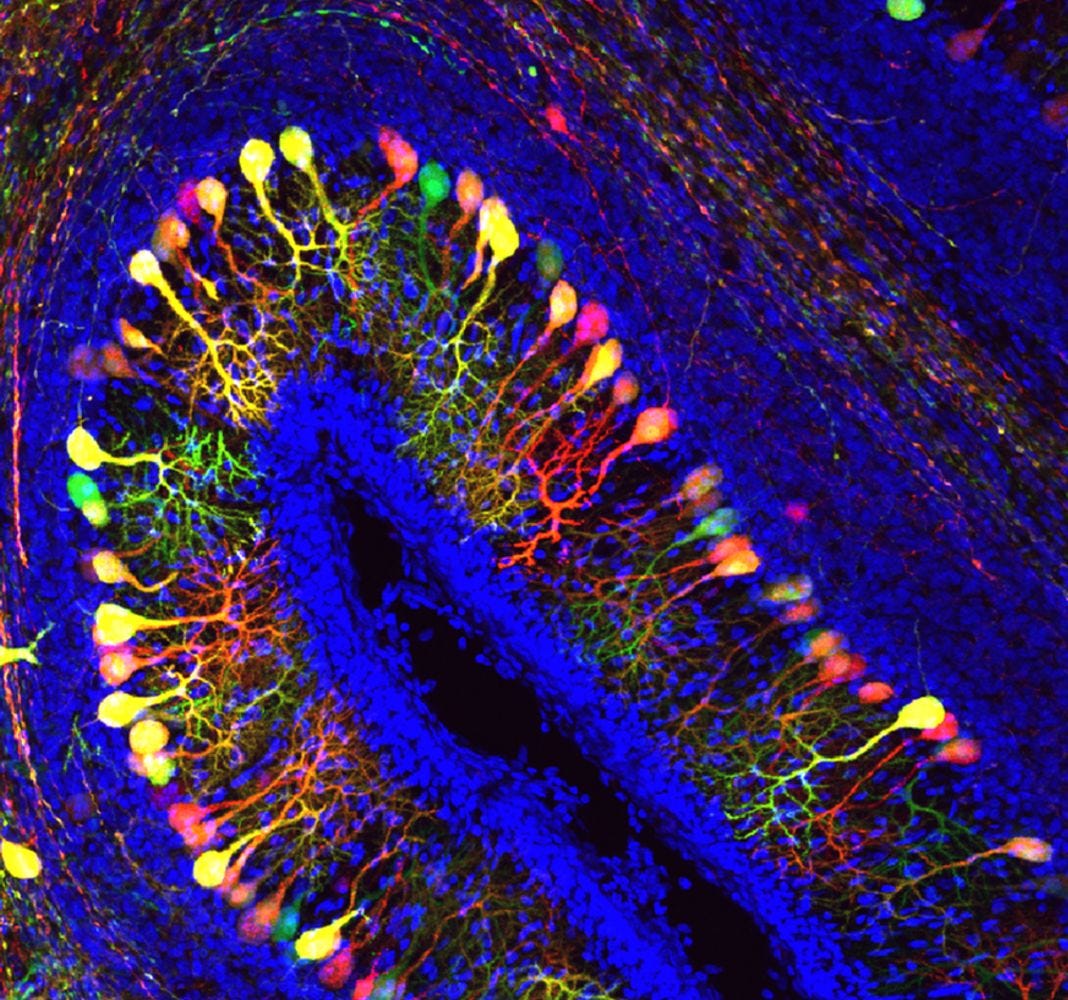
Fredrik Johansson is an excellent neuroscientist at Lund University. Johansson’s ferret experiment seems to indicate that Purkinje cells contain something incredible within them, and so molecular biologists should definitely pay attention to Johansson’s work.
Suppose that Purkinje cells actually contain what Johansson’s experiments seem to indicate that these neurons contain—in that case, any molecular biologist who follows the “ball of thread” that Johansson has given us will find a genuine scientific revolution. You can hardly imagine a more exciting scientific scenario than that, which is why this topic is so fun to look into! But the trick is to get molecular biologists’ attention, since molecular biologists are the ones who are able to follow the “ball of thread” into the Purkinje cell.
Before you read further, make sure to read these two pieces that I’ve published on this topic:
“Is This the Most Interesting Idea in All of Science?” (12 April 2021)
“Are YOU a Molecular Biologist?” (16 July 2021)
I was honored/thrilled to interview Johansson. See below my interview with Johansson that I edited for flow and added hyperlinks to.
Please make sure to subscribe if you like my pieces because that means a lot to me and helps me to thrive on Substack! Enter your email and click this button—that way you’ll get an email whenever I publish a piece:
1) What are the most exciting projects that you’re currently working on?
My time is entirely dedicated to learning as much as possible about how Purkinje neurons learn temporal intervals. There are two exciting projects right now. The first is trying to reproduce the learning phenomenon in a brain slice in order to allow for manipulations that are near-impossible in vivo. The second is an experiment that relates to the fact that several aspects of behavior depend on arithmetic relationships between remembered intervals. Animals perform computations (on stored intervals) like comparing and adding, and these computations’ brain implementation is entirely unknown.
Perhaps surprisingly, several behavioral experiments show that the ratio between the CS–US interval (for example, the time between tone and air puff) and the US–US interval (the time between repetitions) is the causal variable in the appearance of the conditioned response. Explaining those findings seems to require the different intervals in a behavior-conditioning protocol to be stored in a computationally accessible form—and that includes the CS–US interval of a few hundred milliseconds as well as the US–US interval that can be a couple of orders of magnitude greater. The intact animal appears to do this.
And you can design an experiment from which you can derive whether Purkinje neurons also track the longer interval—the jury is out, but it would be remarkable if they do because it would imply even more complex cellular machinery.
2) What does the ferret experiment show?
In my mind, it shows that the Purkinje neuron learns the temporal relationship between two incoming stimuli. The implication is that the cell has a timing mechanism inside—and also a memory substrate inside the cell itself that somehow encodes interval durations.
This is important because it could mean that the hunt to find memory’s physical basis might require us to look inside neurons and that the vast majority of people on this hunt are looking in the wrong place when they look at circuit-level synaptic plasticity.
3) How does the ferret experiment work?
We know from decades of experiments that a small set of eyeblink-controlling Purkinje neurons (in a particular zone of the cerebellum) will—at the end of an eyeblink-conditioning experiment—produce an acquired response that drives the appropriately-timed learned blink. The conditional stimulus (CS) lasts for (e.g.) 300 ms and is followed by an unconditional stimulus (US). In a standard behavioral experiment, the CS is a tone and the US is an air puff directed to the eye. After a few hours of repetition, the animal will produce an anticipatory blink just before the US.
But you can—instead of using these peripheral stimuli—directly stimulate the so-called mossy fibers and the so-called climbing fibers that enter the cerebellum and that are known to signal (respectively) the tone and the air puff. You’ve now reduced the system to the cerebellum.
The Purkinje neurons will still acquire timed responses that can drive the eyeblink. Virtually all theorizing says that that’s achieved based on network effects in the cerebellar cortex’s input layer where—for various hypothesized reasons—different input neurons become active at different times during the elapsing target interval. You could say that the idea is that 1000s of neurons together create a “time code”—or a temporally patterned stream of inputs to the Purkinje neuron—and that an association then forms between the Purkinje cell and the input neurons that happen to be active at the interval’s end. This theorizing is a neat workaround that avoids the problem that we can’t explain how known mechanisms could explicitly numerically encode the duration anywhere.
But we did an experiment that went one step further and bypassed the network of input neurons. To deliver the CS and the US, our experiment stimulated the presynaptic fibers that directly lead to the Purkinje cell—no other cells pre-processed the signals. To deliver the CS, we stimulated the so-called parallel fibers (the input layer neurons’ axons) that lead immediately to the Purkinje neuron. And to deliver the US, we stimulated the so-called climbing fibers that lead to that same Purkinje neuron. In other words, we gave the Purkinje cell—without intervention—the onset/offset signals for the target interval.
And remarkably, the neuron learns to respond at the right time—even in this minimal circuit of one cell and two types of presynaptic fibers. The experimenter can choose a target interval within the 100-millisecond range and the Purkinje cells learn that interval. Having learned an interval, the neuron even adapts when you change the interval—almost like the neuron is directly programmable.
In a nutshell, that’s what all the fuss is about.
4) What do mainstream neuroscientists say when they look at the ferret experiment?
There tends to be a clear gap—at least among the scientists I talk to—between those who are even willing to seriously consider it with an open mind and those who aren’t. And in my experience, it seems like there’s also a generational gap for this particular finding.
I believe that it’s a fair characterization to say that few react in a neutral way—it’s either found very exciting or waved away as impossible.
Divergent findings are almost always met with initial disbelief when there’s a strong consensus theory of how something works—it’s very hard to resist intuition and to resist what every textbook says.
5) Which objections (to the ferret experiment’s remarkable implications) do mainstream neuroscientists put forward? How would you respond to these objections?
In short, they imagine that the parallel-fiber stimulation may go backwards to that layer of 1000s of input neurons (granule cells) and that those input neurons then create the temporally patterned stream of inputs—i.e., the “time code”—that all the standard theories assume. Meaning, maybe the Purkinje neuron could—if the signal went backwards to the granule cells—receive information by some path other than the directly stimulated parallel fibers. This backward loop could cause granule cells to excite inhibitory interneurons (Golgi cells).
But these neurons don’t signal to the Purkinje neuron—they inhibit the granule cells that are the parallel fibers’ source. So this appears to be a dead end. There’s no known way for a backwards signal in the stimulated parallel fibers to reach a population of unstimulated parallel fibers—you would have to postulate an unknown pathway.
To rescue the original theory, you could say—as many do—that the stimulation of intended parallel fibers must activate a bunch of other things, so that a loop somehow happens. And you could also say that the intentionally stimulated fibers have a slight unintended temporal variation that could explain parts of the phenomenon.
But these proposals revert to long-term depression (LTD) of synapses as the acquired physical change, and that just comes with a whole other set of problems in this context. And there’s another problem. For the cell to learn, every CS–US presentation (and the cell needs hundreds) would have to somehow involve the exact same accidental and unintentional activation of other fibers.
But imagine that all this happens. Even then, the CS is a 100 Hz stimulus train—a pulse is delivered every 10 ms—that lasts for a few 100 ms. So presumably the direct intentional stimulation every 10 ms overrides any potential accidental activity due to the train’s previous pulse.
In my mind, there are also plenty of technical details—and additional findings—that make this alternative explanation far-fetched.
In general, those willing to engage and to come this far will accept these points and will accept that their intuitive original objection is hard to defend. But many will still not believe the result. This finding is so unusual that a common reaction is: “I don’t know what—or how—but there must be some other way.”
You could also object that the parallel-fiber stimulation directly excites interneurons in the superficial part of the cerebellar cortex that then inhibit the Purkinje cell.
But we did pharmacological experiments that blocked this input, and there was no effect. And these neurons are clearly not the ones architecturally designed to receive the CS and US signals—the Purkinje neuron is—so why would the learning take place there? And even if that’s where the learning happens, then the same surprising implication would still apply, except for another cell.
6) If Purkinje cells store intervals, then does that mean that every neuron in the brain stores acquired information? And what kinds of acquired information?
Randy Gallistel—who you interviewed before on this topic—would say every neuron.
But in my view, it’s not necessarily every neuron. Certainly there are neurons where you don’t have to presume complex information storage. For example, it makes perfect sense for some neurons to be relay cells that simply receive information and that simply distribute information to various target neurons—for example, a relay cell might receive skin-touch information and send that information to multiple other cells. And it also makes perfect sense for some neurons to play a role in signal/noise filters.
It also depends how you define “acquired information”. The “simpler” neurons (like our imagined relay neuron above) certainly appear to use “normal” plasticity mechanisms to tune their properties in response to experience. So that’s a kind of “learning”, but you can view that as different from storing complex information inside the cell.
But if one neuron does it, then why not all neurons, even if there’s no need to presume that that’s the case? The brain has surprised us many times, so we should keep an open mind.
The only certain thing is that the brain stores far more information than we can even begin to explain at this point in time. I don’t dare to speculate about what kinds of acquired information, but maybe it’s indicative to ask: What can you most easily explain with classical networking processing ideas and with associationism (via plasticity mechanisms like LTP)?
And then it seems to me that there’s a divide between qualitative information (recognizing a smell, associating a smell with a feeling, and so on) and quantitative information (distance, time, probability, and so on). The former might not require any complex information to be stored inside neurons because it is a more easily solvable problem for standard mechanisms, whereas the latter might indeed require that. Purkinje neurons appear to store quantitative information (time). Maybe for quantitative information in general we should look inside the neuron, since we’ve looked for a long time in a particular place (network associations) and found very little.
7) Why ferrets?
In our experimental setup, the animals are decerebrated. And the animal can’t feel anything at that point—a disconnected cerebral cortex gives an EEG recording that looks like deep sleep. This means that you can discontinue anaesthesia (isoflurane/propofol) and study the still-intact cerebellum without anesthesia’s influence, which is essential because anesthesia massively disrupts normal signal processing.
We do very long recordings from single neurons (~20 hours is probably our record). Smaller animals like rats and mice can’t (for technical reasons) withstand that procedure. We use male ferrets—in my experience, even the slightly smaller female ferrets are too small.
And the ferret cerebellum’s eyeblink-controlling area (unlike, say, the mouse’s) lies at the surface, which makes the experiment easier, since it’s not deep in a convoluted gyrus.
Decerebrated setups historically used cats, but our lab switched to ferrets in the ’90s—earlier findings made in the cat were relatively easy to transfer to the ferret because the ferret cerebellum is very similar to the cat cerebellum. We could’ve used other species, but ferrets were already being bred in Northern Europe as experimental animals.
8) Are there any ethical problems with the ferret experiment?
There’s nothing particularly unethical about the ferret experiments, so the same arguments apply here that apply to any animal research.
In most experiments, the research animals will suffer to some degree and the research has to justify that suffering.
But our experiments are unusual in that the animals perceived no suffering whatsoever—not even a pin prick. We don’t even touch the animals until they’re asleep. The subject walks by itself into a chamber where it falls asleep (due to a slow inlet of isoflurane gas) and never regains consciousness. The ferrets are—like the vast majority of research animals—sacrificed, but in our experiments they never suffer at all.
9) What was your reaction when you first saw the ferret experiment’s results?
It was certainly a joyous occasion and very satisfying after years of hard work.
It might sound strange, but I wasn’t really surprised. 20 years of previous lab work had made the standard theory more and more far-fetched in my mind—at the very least, these earlier findings made me view the standard theory as unlikely. It was at least an obvious hypothesis to us that the memory resides in the Purkinje cell, and we’d argued this point before these experiments, and the arrows all pointed in that direction, even if it was just a hypothesis. I did the experiments because we thought that the learning takes place in the Purkinje cell—our lab had published in the previous years several indirect findings that made it hard (for me at least) to account for Purkinje cell behavior without this hypothesis. Many scientists probably brushed off these earlier findings as strange anomalies, just as some do with this one.
10) What were the biggest difficulties regarding the ferret experiment?
It’s not one specific thing that makes these experiments hard—the problem is that many small details have to be perfect all at once.
It’s difficult to perform a flawless surgery. Unlike most of the similar learning experiments where you can record from Purkinje cells that are just slightly deeper into the tissue, these particular experiments restrict you to the more-sensitive surface where it’s easy to damage the very fragile parallel fibers—you can’t go deeper into the tissue to specifically stimulate parallel fibers because there’d be no way to know that you’re not also stimulating the axons of a bunch of unintended cell types.
Then you have to find the right parallel fibers to stimulate, which is far more difficult than it sounds. You can be micrometers off.
And you can’t see the cells or the fibers in vivo—it’s not like when you have a brain slice under a microscope.
So the whole pre-experiment process could take 10 hours of surgery, surface mapping (to find the particular research animal’s eyeblink-controlling area), electrode placement, and—before you’re even in a position to start an experiment—searching for the correct Purkinje cells.
And then once the experiment starts, it’s also difficult to record from the same neuron for many hours in vivo—the brain’s butter-like consistency isn’t very stable.
So it’s not one thing. Any in vivo electrophysiology is difficult. As for the ferret experiments, it’s just the most difficult iteration of what we do.
11) How did the ferret experiment get funded and how expensive was it?
Our lab is mostly funded by the tax funded Swedish Research Council and by a few small private foundations in Sweden.
There’s nothing particularly expensive about these experiments. The entire 5-year project probably cost around $750,000 USD ($150,000 USD per year), but more than half of that was the standard salary for someone to do the work. Fairly standard costs for in vivo electrophysiology.
12) Can this memory for interval duration be created in the Purkinje cell in vitro?
I believe so—I hope to publish some initial results on this later in the year.
13) Have you been able to secure molecular-biologist collaborators to help you follow up on your discovery?
Not yet. There could be many different reasons for this—off the top of my head I can think of four reasons.
First, an interested molecular biologist might lose interest in this when faced with skepticism from neuroscientist colleagues who might not have looked into this in any detail—it’s reasonable to be skeptical if PubMed shows 98 articles that say one thing and 2 articles that say another thing.
Second, maybe they want to see additional evidence that these findings hold—they want to be sure that this will be worthwhile for them.
Third, it’s not easy to figure out what exactly one should try to do first.
Fourth, there’s a lack of funding.
14) What is involved in “following the thread” (to use Gallistel’s metaphor)? What experiments would you most like to do in the future, and why those particular experiments?
You have to repeat the experiments with different measurements/manipulations added on top.
One could try to do various things: measure specific changes in mRNA and in protein levels, block protein synthesis, label and observe protein translocation, inhibit certain intracellular signaling cascades. You could also try to manipulate cell-surface receptors and ion channels in more ways than I’ve done so far.
At the moment, my dream experiment is to compare Purkinje cells that have been conditioned with Purkinje cells that haven’t been conditioned and to look for a clear signal in the mRNA transcription or protein expression. That first clue would allow you to identify key molecular players that are involved and determine where to go next.
15) How long will it take to find incredible things once you start to “follow the thread”?
That’s impossible to say—one always tends to be far too optimistic.
The entire puzzle will most likely take at least a decade to complete, but it’s certainly possible that one could find incredible things in 2–3 years if the work were done.
16) How can there be so much contained in a single neuron, such that it might take decades of dedicated work to “follow the thread”?
An article in National Geographic claimed that the brain is the most complex structure that we know of in the universe—I’m not sure how that conclusion was drawn, but it illustrates the point well.
Here we have a situation like a Russian doll—we knew that the brain’s networks were complex, but now it seems like what goes on inside a single neuron is far more complex than anybody (except maybe Randy Gallistel) thought.
But all of this might seem less surprising if you marvel at (for instance) the amazing feats that bees pull off with very few neurons—or if you just really ponder the fact that there can be up to a couple of billion proteins in a single cell.
17) What makes it so hard to “follow the thread”?
There’s no single specific problem—the key is time, patience, and funding.
And the experiments become harder for every additional measurement/manipulation that you add.